Bioprocess operation modes and advanced bioreactor technology
Cell culture, Drug substance, Manufacturing
- The choice of bioreactor technology plays a key role in the development and manufacture of biological drugs, enabling specific functionalities of the active pharma ingredient. Different bioreactors use various mixing technologies suited for different applications, with stirred-tank reactors providing precise control but potentially high shear stress, orbital shaking reactors offering gentle non-shear mixing, and wave bioreactors facilitating bubble-free aeration with gentle agitation.
- Various cell culture methodologies, including batch, fed-batch, perfusion, and continuous culture, each offer unique benefits and challenges. Batch culture is simple and cost-effective but limited in control and duration, fed-batch enhances productivity through periodic nutrient addition, and perfusion maintains high cell viability and product yield by continuously exchanging medium. Despite higher costs and complexity, perfusion and continuous systems, offer significant advantages for industrial applications.
- Despite generating more waste, single-use bioreactors significantly reduce initial investment, maintenance and cleaning needs, minimize cross-contamination risk, and facilitate quick transitions between different processes, increasing overall efficiency and profitability for pharmaceutical companies and contract manufacturing organizations.
Cell culture in bioreactors is a fundamental process in biotechnology, enabling the controlled proliferation of living cells under carefully tailored and sterile environmental conditions. Bioreactors are used in a wide range of applications, from producing therapeutic proteins and vaccines to cultivating stem cells.
Successful cell culture in bioreactors relies on precise monitoring and control of factors like temperature, pH, dissolved oxygen, gas composition, mixing and nutrient supply to optimize cell growth, viability and product yield while ensuring product quality.1 Among the variety of cell culture methodologies, four main approaches stand out: batch culture, fed-batch culture, perfusion culture, and continuous culture. Each method presents unique advantages and challenges and finds diverse applications.2,3
Cell culture techniques: batch, fed-batch, perfusion and continuous
Batch culture system
The earliest and simplest form of cell culture is batch culture (Figure 1), which involves the inoculation of cells into a fixed volume of growth medium, without the addition of fresh nutrients during the culture period. Throughout the duration of the run, nutrients are gradually depleted and metabolism by-products accumulate inside the vessel. The advantages of this type of cell culture are its straightforward and easy implementation, lower initial cost compared to other types of cell culture, and minimal equipment requirements. However, batch culture suffers from constrains such as limited control over the cell environment, nutrient depletion and metabolism by-products accumulation, which can hinder cell growth and product formation. Moreover, batch culture is not suitable for long term culture durations. Its most common application is a small-scale production and laboratory research (studies on cell biology and metabolism).4
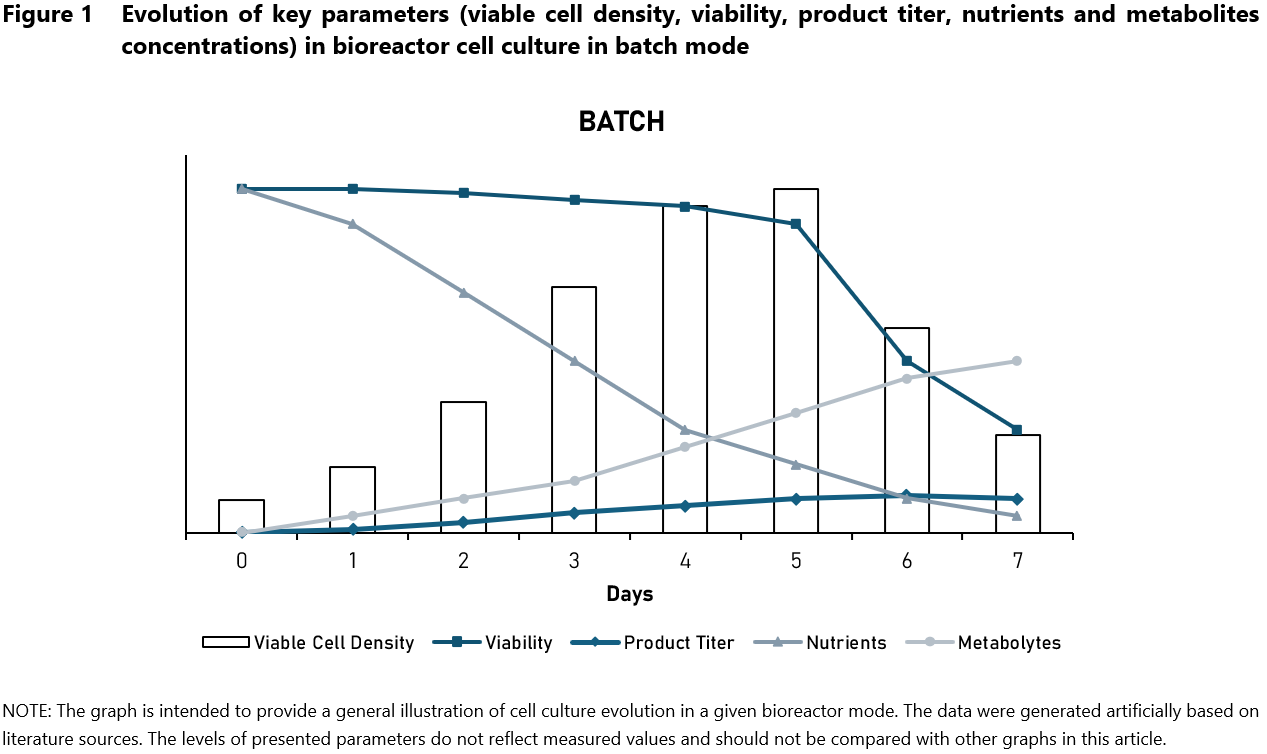
Fed-batch culture system
Fed-batch cultures (Figure 2) are commonly used in industrial applications to enhance cell density and productivity. This technique involves the initial setup of a batch culture, followed by periodic additions of fresh nutrients without removing the culture medium thus the culture volume increases over time. This method helps maintain nutrient levels and control the accumulation of metabolism by-products.
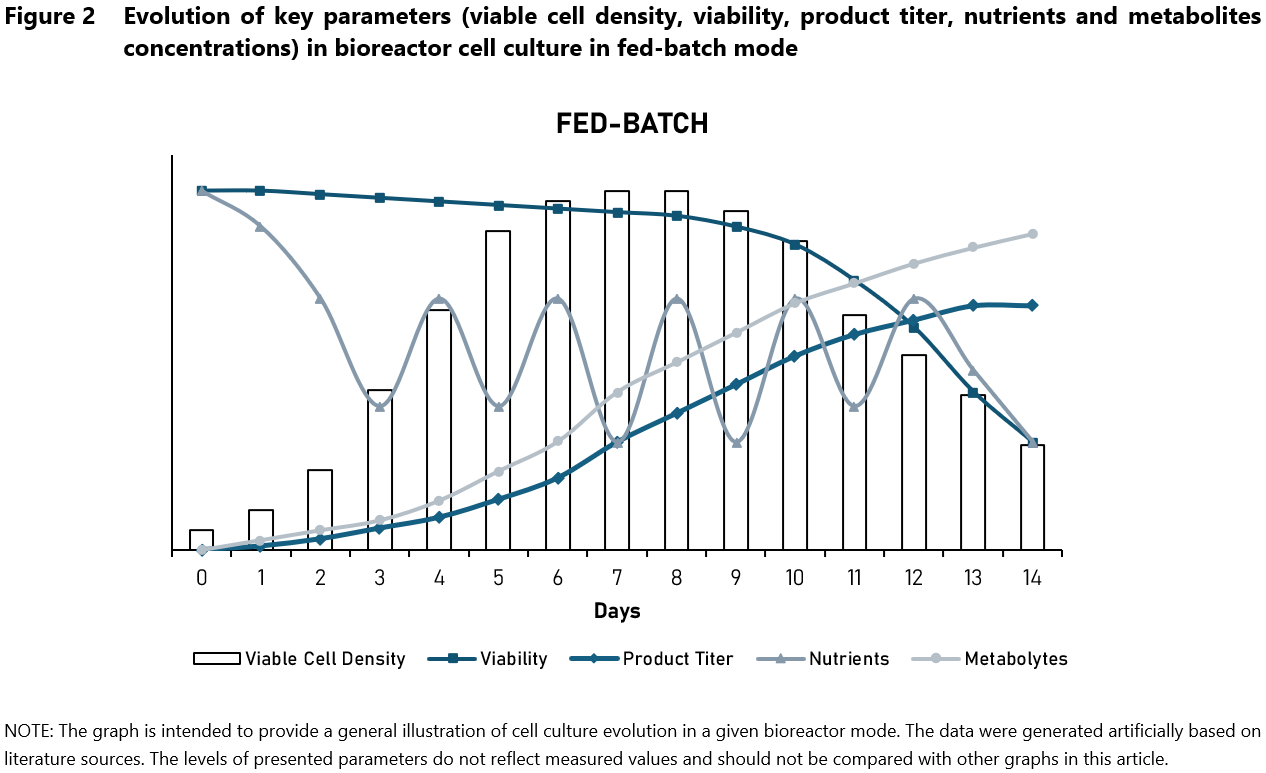
Fed-batch cultures offer several advantages. They provide enhanced control over nutrient levels, improving cell growth and productivity. Additionally, it allows for longer cultivation periods compared to batch cultures, leading to increased cell densities and improved product yield. This cell culture gives us the ability to optimize feeding strategies for different cell lines and products. However, fed-batch cultures also present certain challenges. They require more intricate setup and it is essential to carefully optimize the nutrient feeding strategy to prevent issues such as overfeeding, which can lead to nutrient toxicity, or underfeeding, which can cause nutrient limitation and reduced productivity and even cell death.1,5
Perfusion culture system
Perfusion culture (Figure 3) takes a different approach by continuously removing spent medium and replacing it with fresh, nutrient-rich medium. This continuous exchange sustains optimal nutrient levels and effective removal of metabolism by-products, leading to prolonged high cell viability and sustained production ensuring high product yield. Perfusion culture provides significant advantages for high-value product production by maintaining optimal nutrient levels and metabolites removal, supporting high cell densities and extended culture durations. Perfusion culture requires the use of a system of continuous supply of fresh medium and removal of culture fluid rich in product. It is necessary to use a CRD (cell retention device) module to filter cells and return them back to the bioreactor.
The CRD module can operate on the TFF (tangential flow filtration) or ATF (alternating tangential flow filtration) principle. Perfusion culture is divided into two phases. In the first phase, culture proceeds like a typical fed-batch. When the cells reach the appropriate density, perfusion is started, in which fresh medium is supplied and used medium is removed.
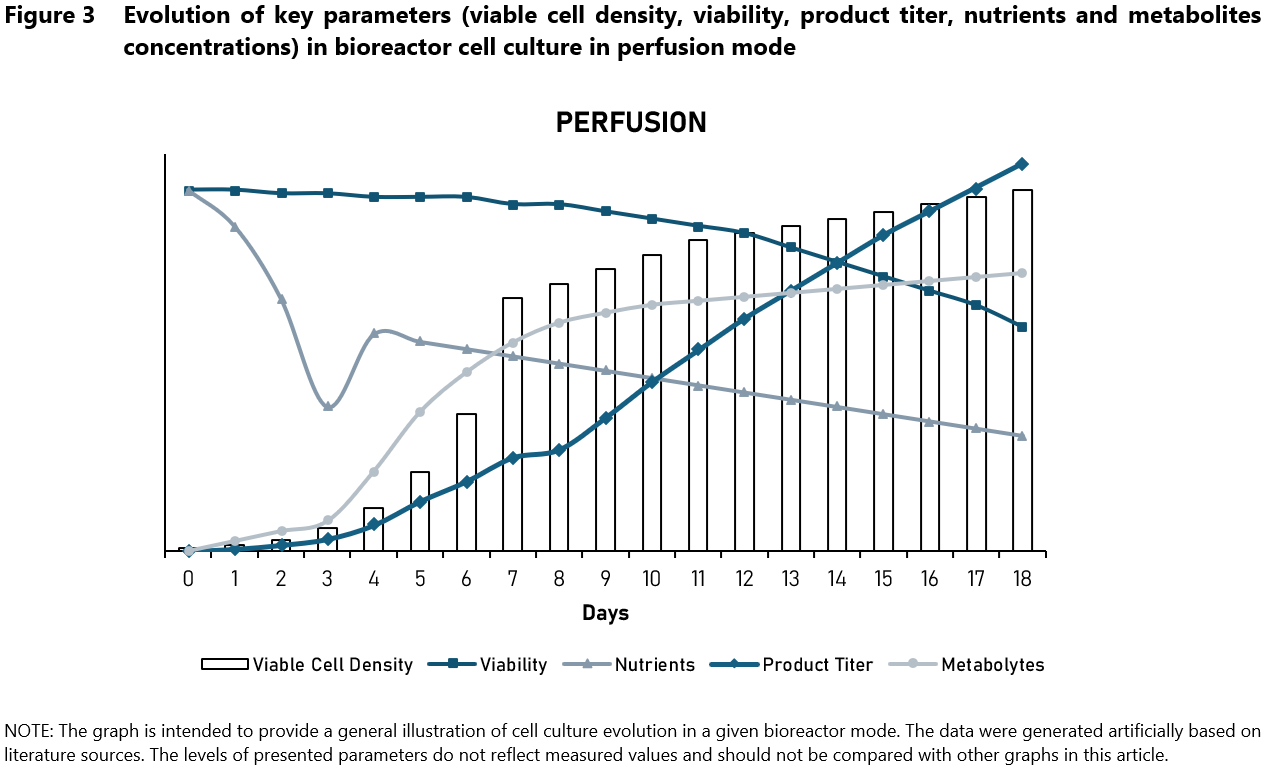
There are two possible approaches to cell retention – returning all cells to the bioreactor or partially removing cells (so-called cell bleed). The choice of approach depends mainly on the growth rate of the cell line and how high the target cell density is. In perfusion culture, cell density is very high, which cannot be achieved in fed-batch mode. These cells produce very large amounts of metabolic by-products, which we cannot completely remove when collecting the used medium, we can only slow down the rate of increase of metabolites. It is possible to manipulate the cell density in perfusion culture by setting a specific cell retention efficiency – the higher the cell retention efficiency, the higher the cell density achieved. It is also possible to control the level of nutrients and metabolic by-products by using an appropriate rate of medium exchange, which is referred to as vessel volume per day (VVD).
Figure 3 represents only one of the possible perfusion culture options – evolution of individual parameters depends on the culture conditions used. Despite the higher initial and operational costs (compared to other types of cell culture) and complexity, the benefits of perfusion culture can outweigh these challenges for specific industrial applications including monoclonal antibodies and therapeutic proteins as well as cell-based therapies.4,6
Continuous culture system
Continuous culture systems (Figure 4) involve the continuous addition of fresh medium while simultaneously removing culture medium containing cells and metabolic waste.
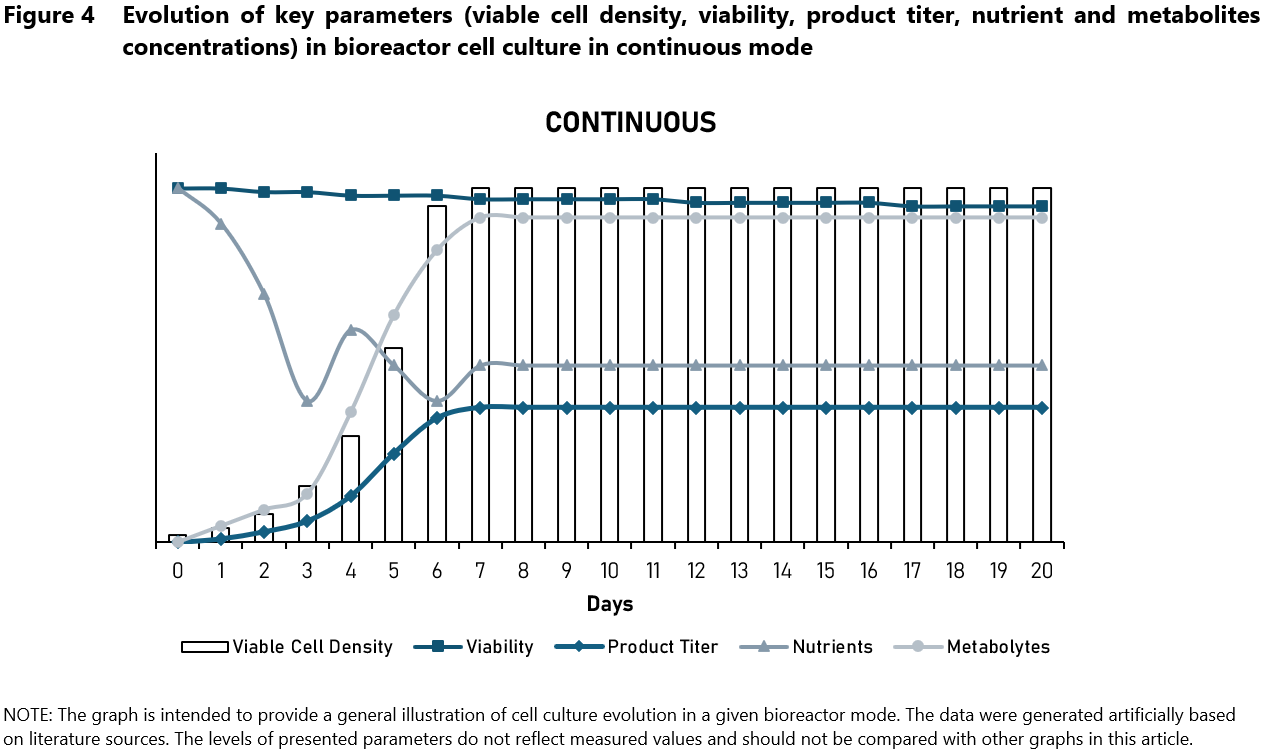
This method allows for the maintenance of cells in a steady-state growth phase (ideal for studying cell physiology), offering precise control over cell growth kinetics and product formation. There are two methods of maintaining cell culture in a steady state: chemostats and turbidostats. Chemostats allow for steady-state concentrations of growth-limiting substrates to be maintained at a fixed level in the culture fluid, which results in highly reproducible ‘steady-state’ growth conditions in which changes in cell density, physiological state, and medium composition of the culture are no longer detectable.7 A turbidostat is a continuous culture system that adjusts the flow rates of the medium and culture removal based on turbidity measurements to keep the cell density constant.
Unlike the chemostat, the nutrient concentrations can fluctuate, but the cell density remains constant.8 Continuous culture systems provide unparalleled productivity and process stability. However, there are challenges associated with continuous culture systems. They require a complex setup and advanced control systems, making them more difficult to manage. Continuous culture system involves significant initial and operating costs. Moreover, this approach may not be suitable for all cell types, particularly those with slow growth rates.
Bioreactor types in biopharmaceutical technology
Another critical aspect of cell culture is the method used to mix and circulate the culture medium within the reactor. Appropriate mixing is crucial in bioreactor operations for several reasons. Effective mixing ensures uniform distribution of nutrients, gases, and other essential components throughout the culture medium, promoting consistent cell growth and maximizing productivity. It also facilitates efficient oxygen transfer from the gas phase to the liquid phase, preventing oxygen limitation and supporting high cell densities and product yields. Additionally, mixing helps maintain uniform pH and temperature, preventing localized fluctuations that could negatively impact cell viability and process efficiency. Managing shear stress resulting from mixing is another critical aspect, as different cell types have varying sensitivities to turbulence; appropriate mixing technology minimizes shear stress, preserving cell integrity and enhancing process robustness. Finally, efficient mixing promotes the dispersion of gases like oxygen and carbon dioxide in the liquid phase, ensuring optimal conditions for cellular respiration, gas exchange, and metabolic activities.9,10
The types of bioreactors used in biopharmaceutical biotechnology include the stirred tank reactor (STR), the wave bioreactor and the orbital shaking reactor (OSR) (Figure 5). Each of them uses a different culture mixing bioreactor technology and has unique advantages for different applications.
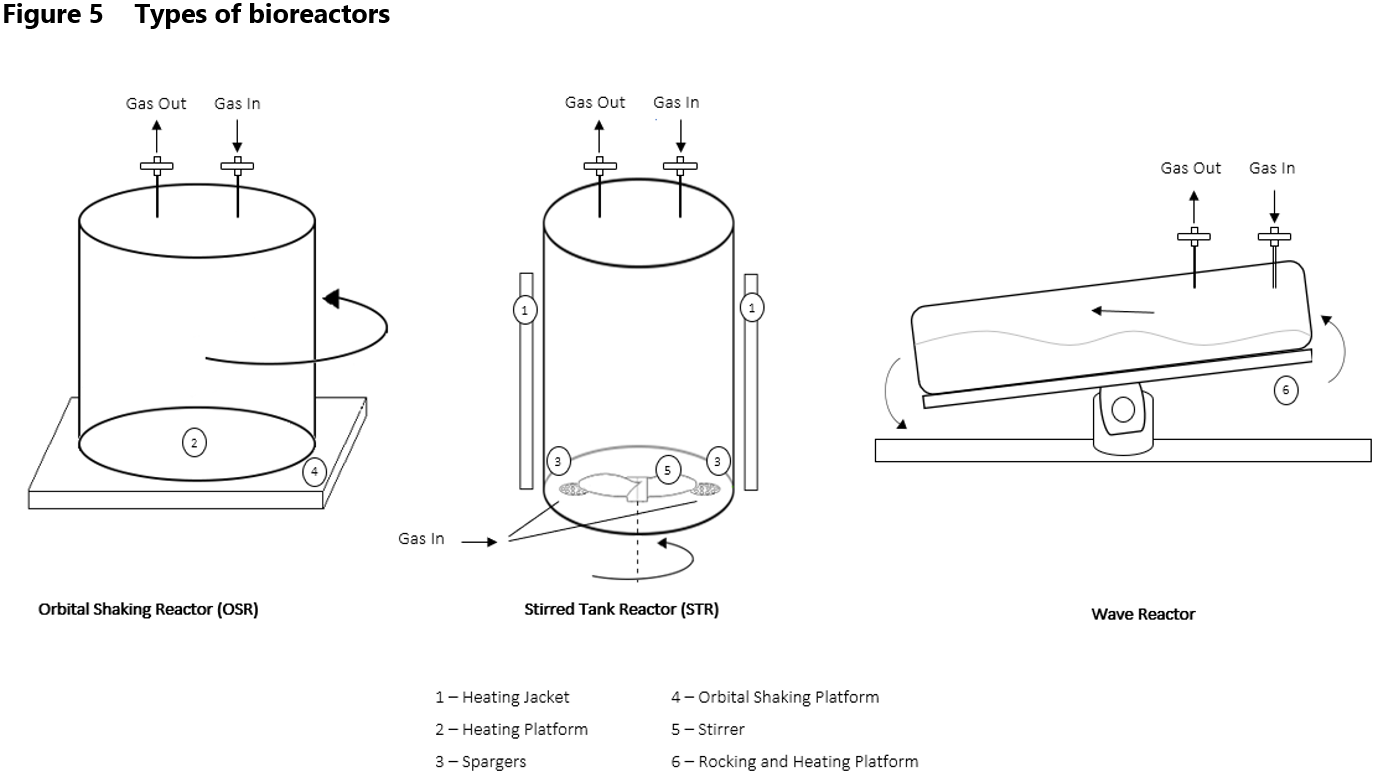
Stirred Tank Bioreactors
Stirred Tank Bioreactors (STRs) are characterized by their cylindrical vessel with a central agitator that mixes the culture medium, ensuring uniform distribution of nutrients, gases, and cells. Due to their advantages, they are widely used for various cell types and applications like large-scale production of therapeutic antibodies, various therapeutic proteins and enzymes as well as vaccines. A central stirring mechanism offer good fluid mixing, oxygen transfer and nutrient distribution.
This bioreactor technology allows precise control of parameters such as:
- temperature,
- pH,
- dissolved oxygen,
- nutrient availability.
pH is monitored using sensors, bioreactors are usually equipped with automatic systems that add acids or bases as needed to control it. Dissolved oxygen levels are detected by sensors and regulated by sparging, which involves introducing air or pure oxygen into the bioreactor. Spargers are essential components of STRs used to introduce gases into the culture medium. They ensure efficient gas-liquid mass transfer by dispersing fine bubbles throughout the medium. The speed of agitation is also adjusted to ensure optimal oxygen distribution. Together, these methods maintain the necessary conditions for cell growth and productivity. However, STRs have potential to generate high shear stress during mechanical agitation, which can be detrimental to sensitive cell cultures. This can lead to cell damage or reduced viability.11
Orbital Shaking Bioreactors
It’s important to note that not all mechanical mixing involves traditional stirrers. The Orbital Shaking Bioreactors (OSRs), use alternative mechanical agitation methods, orbital shaking, instead of conventional impellers. This innovative bioreactor technology approach still achieves effective mixing without the use of stirrers, making it suitable for applications where gentle, non-shear mixing is essential.12 In wave-mixed bag bioreactors, a one – or two-dimensional movement of the bioreactor’s platform induces a wave in the sterile plastic bag containing culture medium and cells. This movement facilitates mixing while continuously renewing the surface of the culture medium and accomplishing bubble-free surface aeration.13
Wave Bioreactors
The Wave Bioreactor system offers several advantages. Its rocking motion provides gentle agitation, reducing shear stress on cells and enabling the cultivation of delicate cell lines. This bioreactor technology is appropriate for smaller operating volumes. They are especially used in the bioprocesses development and optimization, production of cell-based therapies and early-stage manufacturing like a pilot-scale production of biologics.3 However, its rocking motion may not be suitable for all cell types, and oxygen transfer rates can be lower compared to stirred-tank bioreactors, limiting cell densities and productivity in some applications. Despite its limitations, the Wave Bioreactor remains a versatile and widely-used tool in bioprocessing, particularly for applications that benefit from its disposable and gentle mixing capabilities.
Stirred Tank Reactors and Orbital Shaking Reactors are easily scalable, allowing for cell culture at both laboratory and production scales.7 In case of wave-mixed bioreactors, there are not as many volume options available as for STR and OSR, especially on the production scale.
Innovations in bioreactor technology are increasing the prospects for a more sustainable and healthier future. Producers, eager to differentiate themselves in the market, are offering alternatives with specific properties. Bioreactor design is constantly in development. For example, the multi-compartment bioreactor uses technology that allow different cell types or conditions to be introduced into the same reactor. They are used to co-culture different cell types or create gradient environments to simulate in vivo conditions.
Advantages of single-use bioreactor technology in cell culture
All of the types of cell culture described above can be carried out in bioreactors using single-use technology. Currently, most biopharmaceutical companies producing therapeutic proteins use single-use bioreactors, whether STR, OSR or Wave bioreactors. Instead of a culture vessel made from stainless steel or glass, a single-use bioreactor is equipped with a disposable bag. The disposable bag is usually made of a four or five-layer plastic foil. The outer layer is made from PE (polyethylene) or nylon to provide mechanical stability. Inside there may be two/three layers made of EVA (ethylene-vinyl acetate) or EVOH (ethylene-vinyl alcohol), which provides extremely low gas permeability. Finally, a contact layer is made from ULDPE (ultra-low density polyethylene).14
Compared with conventional stainless steel bioreactors, the single-use solutions have some advantages. They provide a range of sizes, configurations, and customization options, allowing for optimization of production and easy adaptation to changing production needs. Single-use bioreactors system are cost-effective due to lower initial capital investment, elimination of extensive maintenance and cleaning measures, and increased productivity and output, leading to enhanced revenue and profits. It also reduces the risk of cross contamination and enhances the biological and process safety. While single-use solutions generate more waste, they significantly reduce water, energy, and chemical usage compared to traditional stainless steel equipment.15
A major reason single-use bioprocessing is popular with pharmaceutical companies and contract manufacturing organizations (CMOs) is because a process area/facility can quickly change from one process (product) to another. This is due to, as stated previously, validation procedures required to prevent microbiological and cross-contamination between products. This increases productivity and profits due to less resources and time being required for each process batch.16,17
Summary
The successful implementation of bioreactor technology depends on precise control of environmental conditions to optimize cell growth and product yield. Among the diverse cell culture methodologies – batch, fed-batch, perfusion, and continuous cultures – each offers distinct benefits and challenges making them suitable for different applications. Stirred Tank Bioreactors (STRs), Orbital Shaking Reactors (OSRs), and Wave Bioreactors use different mixing technologies to meet specific cell culture needs. Effective mixing, nutrient management, and waste removal are critical for maintaining high cell viability and productivity. By understanding and leveraging these bioreactor technologies, bioprocess engineers carefully select the most suitable agitation mode and cell culture methodologies to optimize cell growth, product yield, and the overall success of bioreactor cultures.
Prepared by:
Olga Rojewska
Marcin Tęczar
References
- Pörtner, R. “Bioreactors for mammalian cells.” In: Animal Cell Culture, Al-Rubeai M. (ed.) Springer International Publish. (2015): 89-135.
- Whitford, W. G. “Fed-batch mammalian cell culture in bioproduction.” BioProcess International 2006; 4: 30-40.
- Jyothilekshmi, I., Jayaprakash, N. S. “Trends in monoclonal antibody production using various bioreactor systems.” Journal of Microbiology and Biotechnology 2021; 31(3): 349.
- Suttle, A., Sha, M. “A Beginner’s Guide to CHO Culture: Bioprocess Modes–Batch, Fed-Batch, and Perfusion.” Cell & Gene. Available at: https://www.cellandgene.com/doc/beginner-s-guide-cho-culture-bioprocess-modes-batch-fed-batch-and-perfusion-0001
- Cacciuttolo, M. “Perfusion or fed-batch? A matter of perspective.” Cell culture and Upstream Processing. Taylor & Francis, (2007): 187-198.
- Walther, J., Lu, J., Hollenbach, M., Yu, M., Hwang, C., McLarty, J., Brower, K. “Perfusion Cell Culture Decreases Process and Product Heterogeneity in a Head-to-Head Comparison With Fed-Batch.” Biotechnology Journal 2019; 14(2): e1700733.
- Schaechter, M. (ed.) “Encyclopedia of Microbiology”. Academic Press, 2009.
- Winder, C. L., Lanthaler, K. “The use of continuous culture in systems biology investigations.” Methods in Enzymology 2011; 500: 261-275.
- Tissot, S., Oberbek, A., Reclari, M., Dreyer, M., Hacker, D. L., Baldi, L., Farhat, M., Wurm, F. M. “Efficient and reproducible mammalian cell bioprocesses without probes and controllers?” New Biotechnology 2011; 28(4): 382-390.
- Klöckner, W., Büchs, J. “Advances in shaking technologies.” Trends in Biotechnology 2012; 30(6): 307-314.
- Warnock, J. N., Al-Rubeai, M. “Bioreactor systems for the production of biopharmaceuticals from animal cells. Biotechnology and Applied Biochemistry 2006; 45(Pt 1): 1-12.
- Zhang, X., Stettler, M., De Sanctis, D., Perrone, M., Parolini, N., Discacciati, M., De Jesus, M., Hacker, D., Quarteroni, A., Wurm, F. “Use of orbital shaken disposable bioreactors for mammalian cell cultures from the milliliter-scale to the 1,000-liter scale.” Advances in Biochemical Engineering/Biotechnology 2009; 115: 33-53.
- Eibl, R., Werner, S., Eibl, D. “Bag bioreactor based on wave-induced motion: characteristics and applications.” Advances in Biochemical Engineering/Biotechnology 2009; 115: 55-87.
- Barbaroux, M., Sette, A. “Properties of Materials Used in Single-Use Flexible Containers: Requirements and Analysis”. BioPharm International 2006; 6: 18-29. Available at: https://www.biopharminternational.com/view/properties-materials-used-single-use-flexible-containers-requirements-and-analysis
- Crommelin, D. J. A., Sindelar, R. D., Meibohm, B. (eds.) “Pharmaceutical Biotechnology: Fundamentals and Applications” Springer International Publish (2023).
- Rogge, P., Mueller, D., Schmidt, S. “The single-use or stainless steel decision process: a CDMO perspective.” BioProcess International 2015; 13(11): 10-15. Available at: https://www.bioprocessintl.com/single-use/the-single-use-or-stainless-steel-decision-process-a-cdmo-perspective
- Selden, C., Fuller, B. “Role of Bioreactor Technology in Tissue Engineering for Clinical Use and Therapeutic Target Design.” Bioengineering (Basel) 2018; 5(2): 32.
Related resources
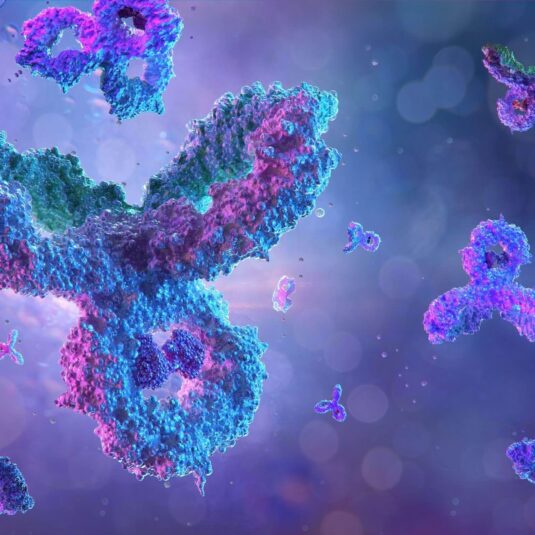
Antibodies Development Best Practices for CDMO Collaboration
Drug development, Manufacturing, Monoclonal antibody
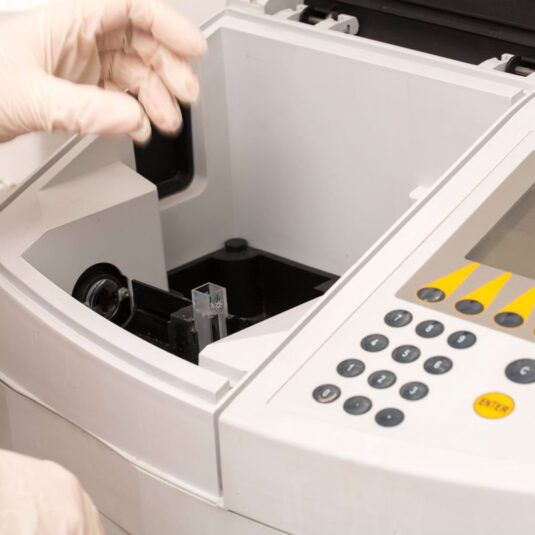
UV-VIS Spectrometry for Protein Concentration Analysis: Principles and Applications
Analytics, Biologics, Proteins
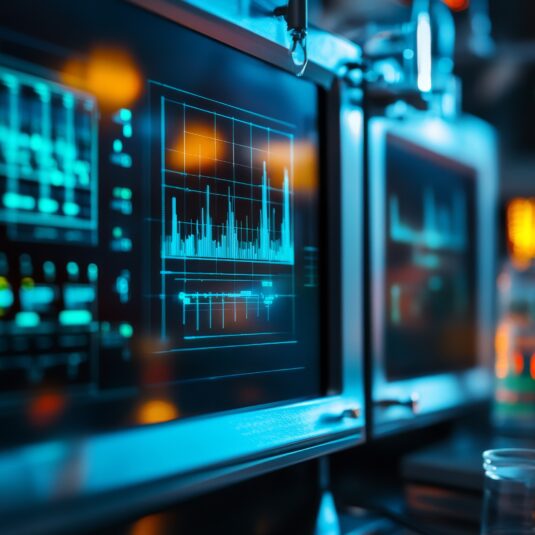
Qualitative analysis of Host Cell Proteins using mass spectrometry
Analytics, Drug development, Drug product, Drug substance